Genomics: Insight

CRISPR-CAS9: a Revolutionary Therapy for Sickle Cell Disease
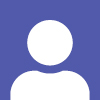
Hypothesis: The gene editing technology CRISPR-Cas9 offers a curative treatment for sickle cell disease, targeting the root cause rather than its symptoms.
What is Sickle Cell Disease?
Sickle cell disease (SCD), an inherited monogenic disorder, affects adult hemoglobin, HbA, a tetrameric protein composed of two alpha globin and two beta globin subunits (Figure 1). HbA is essential for oxygen binding and transport throughout the body (Kato et al., 2018). SCD results from a point mutation in the DNA sequence of the beta-globin gene, HBB. The change in nucleotides results in glutamic acid, a hydrophilic amino acid, being replaced by valine, a hydrophobic amino acid, at the sixth position of the beta globin chain (Mboowa et al., 2024). Hemoglobin’s structure changes, becoming insoluble in red blood cells, which impairs its ability to bind and transport oxygen. As a result, the abnormal hemoglobin, HbS, polymerizes due to the replacement of glutamic acid, a hydrophilic amino acid, with valine, a hydrophobic one, causing red blood cells to sickle (Kato et al., 2018; Figure 1). The distorted shape of the red blood cells further promotes polymerization, making the sickled red blood cells fragile and prone to hemolysis, leading to a decrease in the number of red blood cells that are able to transport oxygen, a condition known as anemia. Polymerization can also result in blockages of blood vessels or capillaries, leading to painful episodes known as vaso-occlusive crises. Furthermore, the blockages prevent red blood cells from delivering oxygen to tissues, leading to irreversible organ damage (Kato et al., 2018). Approximately 300,000 people are affected by SCD annually. Current treatments include pain management through prescription painkillers, such as morphine, and other therapies; however, those treatments are symptom-focused rather than targeting the root cause. (Frangoul et al., 2020).

Created in https://BioRender.com
Figure 1. Different Hemoglobin Structures.
What is CRISPR?
CRISPR/Cas9, a new gene editing technology, enables precise modifications of specific genes within living cells, presenting an approach to treating genetic diseases such as SCD (Liao et al., 2024). In a general sense, the process would involve harvesting stem cells from the patient’s bone marrow, editing the mutated gene to enable the cells to produce functional hemoglobin when reintroduced into the patient’s body. Although the sickle cells are not removed from the patient’s body, inserting the corrected cells allows the body to produce normal hemoglobin instead. The enzyme, Cas9, and a guide RNA are required for CRISPR. The guide RNA directs the enzyme to the target location on a DNA sequence where the gene is to be cleaved (Dance, 2015; Wen & Zhang, 2022). After the disruption of the DNA sequence, the cell repairs it using one of two mechanisms. Homology Directed Repair (HDR) uses an introduced non-mutated DNA sequence as a template to replace the missing sequence. The other method, Non-Homologous End Joining (NHEJ), imprecisely reconnects the two pieces of DNA, altering the targeted gene (Bauer & Orkin, 2015; Dance, 2015; Liao et al., 2024).
Fetal Hemoglobin as a Route for Therapy
It might appear that the direct solution for treating SCD would be simply correcting the genetic mutation by using CRISPR with HDR to edit HBB. That approach would allow the mutated sequence in HBB to be cut and corrected by copying a non-mutated HBB sequence from an introduced unaffected piece of DNA (Liao et al., 2024). Hematopoietic stem cells (HSCs), which are immature cells capable of growing into any type of blood cell, can be modified using CRISPR/Cas9, when working with SCD. However, one of the primary challenges with using HDR is that it relies on cells actively engaging in the cell cycle, specifically during DNA replication, and HSCs are mostly in a resting state where they are not replicating, making them largely resistant to HDR (Bauer & Orkin, 2015).
An alternative approach to using genome editing to address SCD focuses on activating the expression of fetal hemoglobin, HbF. Fetal hemoglobin, present during fetal development, is composed of two alpha and two gamma globin subunits (Figure 1). Unlike HbS, HbF has a higher affinity for oxygen and does not polymerize because the SCD mutation targets beta-globin subunits. Elevated HbF levels, naturally present in some individuals with SCD, are linked to milder disease outcomes. Targeting regulatory genes that increase fetal hemoglobin expression helps compensate for defective beta-globin (Sankaran & Orkin, 2012), supporting the development of treatments aimed at enhancing HbF production as a potential cure for SCD.

Created in https://BioRender.com
Figure 2. Repressive Activity of BCL11A. BCL11A inactivity leads to HbF production.
Genome-Wide Association Studies Discover a Way to Increase HbF
Genome-wide association studies (GWAS) compare genomic DNA sequences among sets of individuals to identify genetic markers linked to specific diseases. They focus on single nucleotide polymorphisms (SNPs), single nucleotide changes in DNA sequences that help scientists locate genes associated with human traits and diseases (Bauer et al., 2013; Uda et al., 2008). Regarding SCD, GWAS identified SNPs connected to reduced symptom severity and detected some located on BCL11A, a gene encoding a transcription factor that silences gamma-globin (Uda et al., 2008; Figure 2). While structurally similar to HbA, HbF differs by incorporating gamma globin instead of beta globin (Figure 1). In patients with SCD, these SNPs disrupt binding of transcription factors to enhancer regions of BCL11A. As a result, the likelihood of BCL11A being expressed decreases, reducing its repressive activity, and increasing HbF levels (Bauer et al., 2013; Figure 2). Thus, inactivating BCL11A represents a potential approach for curing SCD. To test the link between BCL11A and HbF production, scientists used genetically modified mice that expressed human hemoglobin genes to model the switch from gamma-globin to beta-globin. Researchers inhibited the activity of BCL11A in the mouse models using genetic knockout techniques, which disrupted the normal developmental switch of hemoglobin. In mice where BCL11A activity was inhibited, the results showed higher levels of HbF accompanied by higher gamma globin expression (Esteghamat et al., 2013; Figure 2).
Using Casgevy to Treat Sickle Cell Disease
Casgevy, a gene therapy that uses CRISPR-Cas9 to treat SCD, involves extracting HSCs from the patient’s bone marrow and knocking out BCL11A to promote fetal hemoglobin production. The edited stem cells are then transplanted back into the patient, where they differentiate and mature into healthy red blood cells (Wen & Zhang, 2022; Wong, 2023).
The development of treatments such as CRISPR/Cas9 has provided significant advancements in combating diseases. One notable success is Victoria Gray, the first patient to receive the Casgevy treatment, who reported significant relief from the chronic pain of SCD eight months after treatment (Stein, 2021).
While a great advancement, there are side effects associated with the CRISPR/Cas9 treatment, with patients experiencing nausea, fatigue, fevers, and becoming more susceptible to infections (Kato et al., 2018). Additionally, NHEJ repair can introduce unintended mutations at cleavage sites, potentially affecting other genes (Asmamaw & Zawdie, 2021). Another challenge is the cost, as gene-editing therapies remain very expensive, with CRISPR/Cas9 costing approximately $2.2 million per patient. Insurance plans may not fully cover these treatments, creating financial barriers for low- and middle-income families and limiting accessibility. It is important to spread awareness of new gene editing technologies like CRISPR, to gain more attention in efforts to provide more research to make the therapy more accessible as well as affordable to everyone.
Looking ahead, research continues to refine gene-editing techniques, with efforts to also improve affordability and expand insurance coverage. The success of early patients like Gray demonstrates the transformative power of CRISPR therapies, offering hope for a future where genetic diseases like SCD can be treated more widely and equitably, to enhance the quality of life for patients.
The success of early patients like Gray demonstrates the transformative power of CRISPR therapies, offering hope for a future where genetic diseases like SCD can be treated more widely and equitably, to enhance the quality of life for patients.
References
- Asmamaw, M., & Zawdie, B. (2021). Mechanism and Applications of CRISPR/Cas-9-Mediated Genome Editing. Biologics: Targets & Therapy, 15(1), 353–361. https://www.ncbi.nlm.nih.gov/pmc/articles/PMC8388126/
- Bauer, D. E., Kamran, S. C., Lessard, S., Xu, J., Fujiwara, Y., Lin, C., Shao, Z., Canver, M. C., Smith, E. C., Pinello, L., Sabo, P. J., Vierstra, J., Voit, R. A., Yuan, G.-C. ., Porteus, M. H., Stamatoyannopoulos, J. A., Lettre, G., & Orkin, S. H. (2013). An Erythroid Enhancer of BCL11A Subject to Genetic Variation Determines Fetal Hemoglobin Level. Science, 342(6155), 253–257. https://doi.org/10.1126/science.1242088
- Bauer, D. E., & Orkin, S. H. (2015). Hemoglobin switching’s surprise: the versatile transcription factor BCL11A is a master repressor of fetal hemoglobin. Current Opinion in Genetics & Development, 33, 62–70. https://doi.org/10.1016/j.gde.2015.08.001
- Dance, A. (2015). Core Concept: CRISPR gene editing. Proceedings of the National Academy of Sciences of the United States of America, 112(20), 6245–6246. https://www.jstor.org/stable/26462797
- Esteghamat, F., Gillemans, N., Bilic, I., van den Akker, E., Cantù, I., van Gent, T., Klingmüller, U., van Lom, K., von Lindern, M., Grosveld, F., Bryn van Dijk, T., Busslinger, M., & Philipsen, S. (2013). Erythropoiesis and globin switching in compound Klf1::Bcl11a mutant mice. Blood, 121(13), 2553–2562. https://doi.org/10.1182/blood-2012-06-434530
- Frangoul, H., Altshuler, D., Cappellini, M. D., Chen, Y.-S., Domm, J., Eustace, B. K., Foell, J., de la Fuente, J., Grupp, S., Handgretinger, R., Ho, T. W., Kattamis, A., Kernytsky, A., Lekstrom-Himes, J., Li, A. M., Locatelli, F., Mapara, M. Y., de Montalembert, M., Rondelli, D., & Sharma, A. (2020). CRISPR-Cas9 Gene Editing for Sickle Cell Disease and β-Thalassemia. New England Journal of Medicine, 384(3). https://doi.org/10.1056/nejmoa2031054
- Kato, G. J., Piel, F. B., Reid, C. D., Gaston, M. H., Ohene-Frempong, K., Krishnamurti, L., Smith, W. R., Panepinto, J. A., Weatherall, D. J., Costa, F. F., & Vichinsky, E. P. (2018). Sickle Cell Disease. Nature Reviews Disease Primers, 4(1). https://doi.org/10.1038/nrdp.2018.10
- Liao, H., Wu, J., VanDusen, N. J., Li, Y., & Zheng, Y. (2024). CRISPR/Cas9-Mediated Homology-Directed Repair for Precise Gene Editing. Molecular Therapy — Nucleic Acids, 35(4), 102344–102344. https://doi.org/10.1016/j.omtn.2024.102344
- Mboowa, G., Sserwadda, I., Kanyerezi, S., Tukwasibwe, S., & Benson Kidenya. (2024). The dawn of a cure for sickle cell disease through CRISPR‐based treatment: A critical test of equity in public health genomics. Annals of Human Genetics. https://doi.org/10.1111/ahg.12558
- Pagliarulo, N. (2023, December 8). Pricey new gene therapies for sickle cell pose access test. BioPharma Dive. https://www.biopharmadive.com/news/crispr-sickle-cell-price-millions-gene-therapy-vertex-bluebird/702066/
- Sankaran, V. G., & Orkin, S. H. (2012). The Switch from Fetal to Adult Hemoglobin. Cold Spring Harbor Perspectives in Medicine, 3(1), a011643–a011643. https://doi.org/10.1101/cshperspect.a011643
- Stein, R. (2021, December 31). First sickle cell patient treated with CRISPR gene-editing still thriving. NPR.org. https://www.npr.org/sections/health-shots/2021/12/31/1067400512/first-sickle-cell-patient-treated-with-crispr-gene-editing-still-thriving
- Uda, M., Galanello, R., Sanna, S., Lettre, G., Sankaran, V. G., Chen, W., Usala, G., Busonero, F., Maschio, A., Albai, G., Piras, M. G., Sestu, N., Lai, S., Dei, M., Mulas, A., Crisponi, L., Naitza, S., Asunis, I., Deiana, M., & Nagaraja, R. (2008). Genome-wide association study shows BCL11A associated with persistent fetal hemoglobin and amelioration of the phenotype of -thalassemia. Proceedings of the National Academy of Sciences, 105(5), 1620–1625. https://doi.org/10.1073/pnas.0711566105
- Wen, W., & Zhang, X.-B. (2022). CRISPR-Cas9 gene editing induced complex on-target outcomes in human cells. Experimental Hematology, 110, 13–19. https://doi.org/10.1016/j.exphem.2022.03.002
- Wong, C. (2023). UK First to Approve CRISPR Treatment for diseases: What You Need to Know. Nature, 623(623), 676. https://doi.org/10.1038/d41586-023-03590-6
About the Author
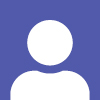
Alla Zurnachyan is a second-year student, majoring in Biology with a minor in Psychology. Mary Cloud Namy is a second-year student majoring in Biochemistry and Molecular Biology. Nii Jarfro Larkai is a second-year student majoring in Biology with a minor in Psychological Science. All three are enrolled at The Pennsylvania State University.
Mentor: Dr. Bryan Wang Affiliation: The Pennsylvania State University